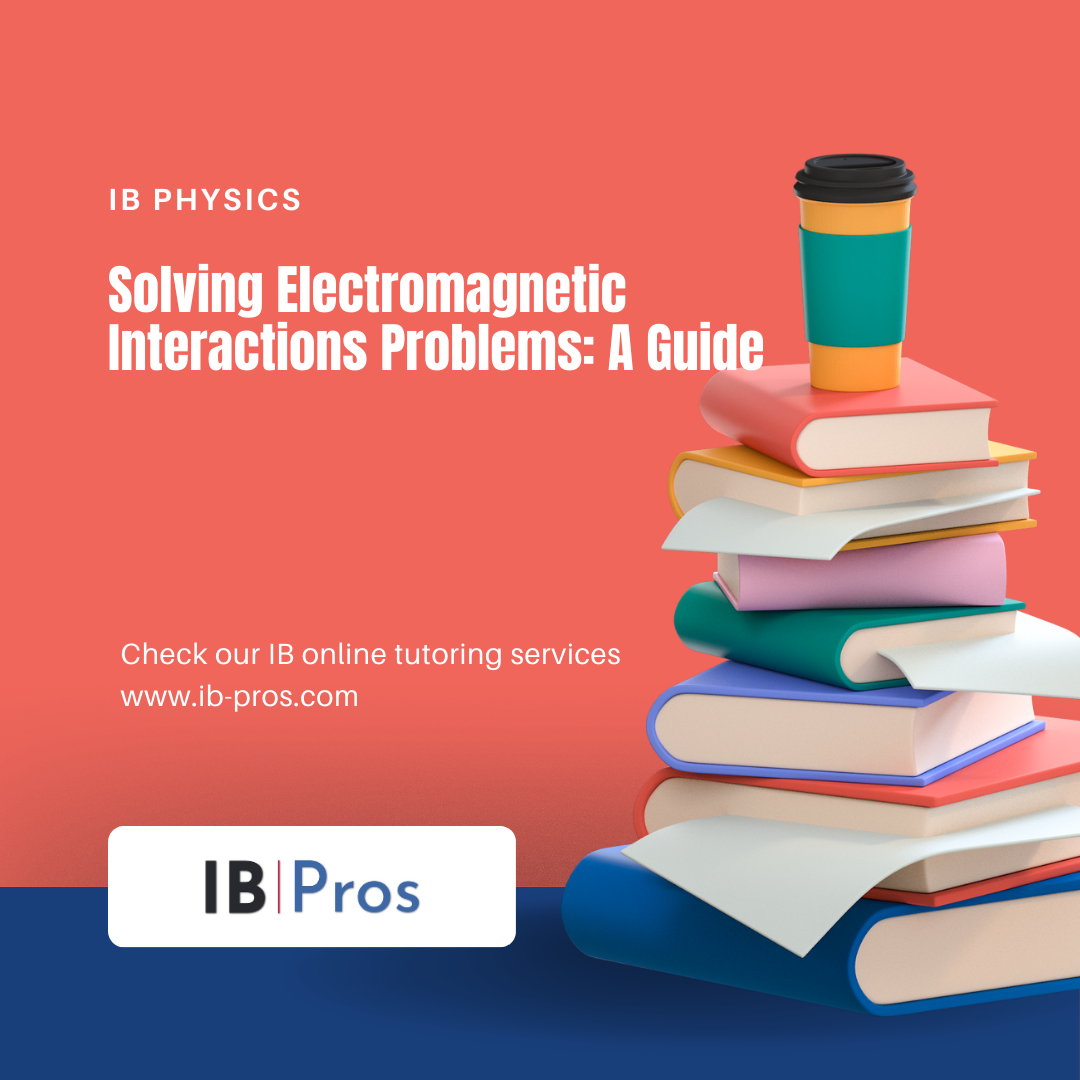
Electromagnetic interactions are at the heart of countless modern technologies, from the transmission of information through radio waves to the intricate dance of particles in quantum computing devices. The complexity of these interactions is governed by fundamental principles and equations that can prove challenging even for seasoned professionals. In navigating the multifaceted landscape of electromagnetic problems, it is essential to have a robust understanding of both theoretical underpinnings and practical applications. As we embark on a comprehensive exploration of this topic, we will dissect the intricacies of Maxwell’s equations, untangle the subtle nuances of boundary conditions, and investigate the perplexing phenomenon of wave interference. Moreover, we will delve into the indispensable realm of numerical simulation techniques, providing the analytical tools necessary to approach these problems with confidence. The subsequent discussion promises to equip the inquisitive mind with the strategies required to address and resolve the sophisticated puzzles posed by electromagnetic interactions, potentially unlocking new avenues of innovation in the process.
Key Takeaways
- Understanding the fundamental principles of electromagnetic interactions, such as Maxwell’s equations and Coulomb’s law, is crucial for analyzing and predicting electromagnetic phenomena.
- Mastery of Maxwell’s equations is essential for solving complex problems involving electromagnetic waves and fields in various applications, including wireless communications and optical systems.
- Boundary conditions, derived from Maxwell’s equations, play a critical role in determining fields at interfaces and are important for modeling electromagnetic phenomena.
- Wave interference, both constructive and destructive, poses challenges in the design and analysis of communication systems and signal processing devices, and understanding it is essential for designing effective systems.
Understanding Electromagnetic Fundamentals
Before delving into complex electromagnetic interactions, it is crucial to establish a firm grasp of the fundamental principles that govern electric and magnetic fields and their interplay. The cornerstone of these principles lies within Maxwell’s equations, which describe how electric charges produce electric fields (E-fields) and how moving charges, or electric currents, give rise to magnetic fields (B-fields). These fields are not merely static entities; they can change with time and propagate through space, leading to the phenomenon of electromagnetic waves.
A precise understanding of the electric field emanates from Coulomb’s law, which quantifies the electric force between two point charges as directly proportional to the product of their charges and inversely proportional to the square of the distance between them. This force is mediated by the electric field, which thus serves as a vector field representing the force a charge would experience at any point in space.
Magnetic fields, on the other hand, are governed by Ampère’s law with Maxwell’s addition, which states that magnetic fields are generated by electric currents and the change in electric fields. The relationship between electric and magnetic fields is further elaborated by Faraday’s law of electromagnetic induction, which explains how a time-varying magnetic field induces an electromotive force and, consequently, an electric current in a closed circuit. Understanding these laws and their implications is imperative for the precise analysis of electromagnetic interactions in various applications.
Mastering Maxwell’s Equations
Maxwell’s equations constitute the cornerstone of classical electromagnetism, providing a comprehensive framework for understanding the behavior of electric and magnetic fields. Accurate analysis of these equations is critical, particularly when applying boundary conditions to solve complex problems involving electromagnetic waves and fields. Mastery of these equations, therefore, is essential for predicting electromagnetic interactions in a diverse array of applications, from wireless communications to optical systems.
Understanding Fields and Waves
To fully grasp the complex nature of electromagnetic phenomena, one must first become proficient in the mathematical framework provided by Maxwell’s equations, which describe how electric and magnetic fields propagate as waves through space and time. These fields and waves are not merely abstract concepts; they have real-world implications and can be quantified using the following elements of Maxwell’s equations:
- Gauss’s Law for Electricity: This equation relates the electric fields to the charges that produce them, indicating the divergence of an electric field is proportional to the charge density.
- Gauss’s Law for Magnetism: It asserts the non-existence of magnetic monopoles; the net magnetic flux through a closed surface is always zero, reflecting the divergence-free nature of magnetic fields.
- Faraday’s Law of Induction and Ampere’s Law with Maxwell’s Addition: These describe how a time-varying magnetic field generates an electric field (Faraday’s Law) and conversely, how a time-varying electric field and electric currents produce a magnetic field (Ampere’s Law, modified by Maxwell).
Understanding and applying these principles is crucial for analyzing and solving complex electromagnetic interactions.
Analyzing Boundary Conditions
When approaching electromagnetic problems, analyzing the boundary conditions is essential for applying Maxwell’s equations effectively in complex scenarios. Accurate characterization of these conditions facilitates the determination of fields at interfaces where mediums with distinct electromagnetic properties converge. Boundary conditions are derived from Maxwell’s equations and reflect the continuity or discontinuity of the normal and tangential components of electric and magnetic fields. The tangential electric field and the normal component of the magnetic flux density must remain continuous across a boundary. Conversely, the normal electric flux density and tangential magnetic field may exhibit discontinuity, proportional to surface charge and current densities, respectively. Mastery of these principles ensures precise modeling of electromagnetic phenomena, crucial for the development of advanced materials and technologies.
Analyzing Boundary Conditions
Understanding the behavior of electromagnetic fields at the interface of different media necessitates a thorough analysis of boundary conditions. This analysis is critical for accurately predicting how fields interact with materials, which is essential in designing electronic devices, waveguides, and other electromagnetic systems. To ensure precision in this process, one must consider the following aspects:
- Continuity of Tangential Components: The tangential components of the electric field (E) and the magnetic field (H) must be continuous across the boundary. This requirement arises from Maxwell’s equations, which dictate that the integral of the electric field around a closed loop and the magnetic field through a closed surface are conserved quantities. Mathematically, this is expressed as ( E_{1t} = E_{2t} ) and ( H_{1t} = H_{2t} ), where subscripts 1 and 2 refer to media on either side of the boundary.
- Normal Component Discontinuities: The normal components of displacement field (D) and magnetic flux density (B) can be discontinuous, depending on the presence of free charges or magnetic materials. These discontinuities are quantified by the relations ( D_{1n} – D_{2n} = sigma_f ) and ( B_{1n} – B_{2n} = 0 ), with ( sigma_f ) representing surface charge density.
- Boundary Impedance Matching: To minimize reflection and maximize transmission of energy, the impedance of the media at the boundary should be matched. This is particularly important in the design of antennas and transmission lines, where impedance mismatch can lead to standing waves and power loss.
These rules form the foundation of boundary condition analysis, enabling engineers and physicists to model and design electromagnetic systems with high fidelity.
Tackling Wave Interference Challenges
Wave interference, a fundamental phenomenon where electromagnetic waves superpose to form a resultant wave, poses significant challenges in the design and analysis of communication systems, sensors, and signal processing devices. The complexity arises due to the constructive and destructive interference patterns that can enhance or diminish signal integrity, respectively. To address these challenges, engineers employ meticulous analytical models and computational simulations to predict and counteract interference effects.
The deployment of multiple signal pathways, utilizing both spatial and frequency diversity, is a strategic approach to mitigate interference. By distributing the energy of a signal across various channels, the probability of significant signal degradation due to interference is reduced. Furthermore, adaptive signal processing techniques, such as beamforming and dynamic frequency selection, are integral in real-time interference management. These methods leverage the capabilities of advanced algorithms to adjust system parameters in response to the instantaneous electromagnetic environment.
On the physical layer, material science plays a pivotal role in developing substrates and coatings that minimize wave reflection and absorption, thus attenuating interference sources. Additionally, the precise arrangement of components within devices to avoid cross-talk and the strategic distancing of systems that may interfere with one another are critical considerations. Collectively, these measures form a robust framework for tackling the challenges of wave interference in complex electromagnetic systems.
Implementing Numerical Simulation Techniques
Numerical simulation techniques are pivotal in predicting electromagnetic interactions when analytical solutions become infeasible. The selection of appropriate simulation software is critical and depends on the specific requirements of the problem, including the frequency range, material properties, and the scale of the electromagnetic phenomena. Once a suitable software is chosen, meticulous definition of boundary conditions and parameters is essential to ensure the accuracy of the results, which must subsequently be rigorously analyzed to validate the model and draw meaningful conclusions.
Choosing Simulation Software
Selecting the appropriate simulation software is critical for accurately modeling and analyzing electromagnetic interactions within various systems and materials. When determining the most suitable software for electromagnetic problem-solving, one must scrutinize several key technical specifications and capabilities:
- Solver Efficiency: The chosen software should possess a robust solver capable of handling complex geometries and materials with high computational efficiency, ensuring accurate results within reasonable time frames.
- Multiphysics Integration: For comprehensive analysis, the software must support multiphysics capabilities, enabling simultaneous consideration of electromagnetic interactions alongside thermal, mechanical, or other physical phenomena.
- User Interface and Usability: An intuitive user interface coupled with advanced scripting options allows for precise control over simulation parameters, facilitating user engagement and streamlining the modeling process.
A meticulous examination of these criteria will guide researchers and engineers to a software platform that aligns with the sophisticated demands of electromagnetic simulations.
Setting Up Parameters
Establishing accurate parameters is the cornerstone of effective numerical simulation in electromagnetic problem-solving, requiring meticulous attention to the physical properties and boundary conditions of the system under study. Parameters must be defined with scientific rigor, ensuring that they align with empirical data and theoretical models. The precision of these parameters directly impacts the validity of the simulation outcomes, necessitating a systematic approach to their determination.
Parameter TypeDescriptionConsiderationMaterial PropertiesElectrical conductivity, permeability, and permittivity.Must reflect the true physical behavior of the materials.Geometrical ConstraintsDimensions and tolerances of the modeled structures.Accuracy here is crucial to the fidelity of the simulation.Boundary ConditionsDefines the limits of the simulation domain.Should capture the interaction with the environment accurately.Source CharacteristicsFrequency, amplitude, and phase of sources.Need to be set to represent the actual operational conditions.
Analytical and empirical validation is paramount to confirm that the chosen parameters are leading to credible simulations.
Analyzing Simulation Results
Having meticulously established the parameters for electromagnetic simulations, the subsequent phase entails a detailed examination of the simulation outcomes using advanced numerical techniques. This analysis is pivotal for interpreting the physical phenomena and validating the simulation model’s accuracy. The following list illustrates the systematic approach:
- Data Visualization: Utilize graphical tools to represent the fields, currents, and charges, discerning patterns and anomalies that warrant further investigation.
- Quantitative Assessment: Perform statistical analysis on the results, comparing numerical data against theoretical predictions or experimental data to measure deviations and establish confidence in the simulation’s reliability.
- Sensitivity Analysis: Investigate the impact of parameter variations on the outcomes, thereby identifying the robustness of the model and the critical parameters that significantly influence the simulation.
Applying Problem-Solving Strategies
To effectively tackle electromagnetic interactions problems, one must first delineate the relevant physical principles and mathematical frameworks guiding the phenomena. This approach requires an analytical mindset that rigorously applies Maxwell’s equations, the Lorentz force law, and boundary conditions. Moreover, the problem-solving strategy must also integrate the use of mathematical tools such as vector calculus and differential equations.
The following table provides a structured approach to the problem-solving process in electromagnetic interactions:
StepActionConsideration1Identify the ProblemDetermine the nature of the electromagnetic interaction and the physical quantities involved.2Formulate EquationsApply Maxwell’s equations and other relevant laws to describe the system mathematically.3Simplify the ProblemUtilize symmetries, approximations, or numerical methods to make the problem more tractable.4Solve the EquationsEmploy analytical or numerical techniques to find solutions to the equations.5Analyze the ResultsInterpret the solutions in the context of the original problem, checking for physical plausibility and consistency.
Employing this structured approach ensures that each aspect of the electromagnetic problem is addressed systematically. This methodology promotes a thorough understanding of the underlying physics, thereby leading to precise and accurate solutions.
Frequently Asked Questions
How Has the Advancement of Machine Learning Affected the Methods for Solving Electromagnetic Interaction Problems?
The advent of machine learning has significantly enhanced the capability to solve electromagnetic interaction problems by enabling the analysis of complex datasets and the identification of patterns that traditional methods may overlook. Sophisticated algorithms can now predict electromagnetic phenomena with greater accuracy, optimize design processes, and reduce computational time, leading to more efficient and innovative solutions in fields such as telecommunications, medical imaging, and aerospace engineering.
What Are the Ethical Considerations When Applying Electromagnetic Technologies in Public Spaces, Such as the Potential Impact on Health or Privacy?
When applying electromagnetic technologies in public spaces, ethical considerations must encompass potential health impacts, such as radiation exposure risks, and privacy concerns, including unauthorized data collection. It is imperative to ensure compliance with safety standards to mitigate health risks and establish stringent data protection measures to safeguard personal information against misuse. These ethical frameworks are crucial to maintain public trust and balance technological advancement with societal well-being.
How Do Electromagnetic Interactions Play a Role in the Development of Wireless Power Transfer Systems, and What Are the Limitations?
Electromagnetic interactions are fundamental to wireless power transfer systems, employing inductive coupling for energy transmission. However, efficiency declines sharply with increased distance between transmitter and receiver, posing a significant limitation. Furthermore, alignment and positioning must be precise to optimize transfer, and there remain challenges in scaling up power for larger devices without compromising safety standards due to potential electromagnetic exposure, indicating a need for ongoing refinement in these systems’ design and implementation.
Can Quantum Mechanics Provide a Deeper Understanding or Novel Approaches to Solving Complex Electromagnetic Problems Beyond Classical Electrodynamics?
Quantum mechanics indeed offers profound insights into electromagnetic phenomena that classical electrodynamics cannot fully address. By considering the quantization of fields and particle-wave duality, quantum approaches enable the examination of phenomena at microscopic scales, where classical theories may fail. Such quantum perspectives are crucial for understanding complex interactions, like those in nano-electronics and quantum computing, and can inspire innovative techniques for tackling intricate electromagnetic challenges.
What Are the Career Prospects and Industry Demands for Professionals Skilled in Solving Electromagnetic Interaction Problems?
Professionals adept in solving electromagnetic interaction problems are highly sought after across various industries, including telecommunications, aerospace, defense, and healthcare. Their expertise facilitates the advancement of wireless technologies, satellite communications, and medical imaging systems. As technology evolves, the demand for such specialized skills is expected to grow, ensuring robust career prospects for individuals with proficiency in electromagnetic theory and its practical applications.
Conclusion
In conclusion, addressing electromagnetic interaction problems necessitates a robust comprehension of the fundamental principles, meticulous application of Maxwell’s Equations, and careful consideration of boundary conditions. Wave interference and numerical simulations present significant challenges that require strategic problem-solving approaches. Mastery in these areas enables the precise analysis and resolution of complex electromagnetic phenomena, contributing to advancements in fields ranging from telecommunications to medical imaging.